Advance Coal Science
How Coal is formed ?
Coal is made from plant material (trees, small bushes and grasses) that have died and been submerged quickly in water. In this process, fungi and bacteria degrade the plant material and form peat. The rate of accumulation of plant material must exceed the rate at which the plant material is consumed by the bacteria and fungi. The rate of decay is a function of temperature and cellulose decomposing bacteria are most active in the range 35 – 40°C under these conditions plant material tends to become homogenised (gelified) where-as peat formed under cold conditions tends to preserve the original plant material in bands. Carboniferous and Permian coal accumulated under cool conditions and consequently the coal is more banded than Cretaceous and Tertiary coals that were formed under much warmer conditions.
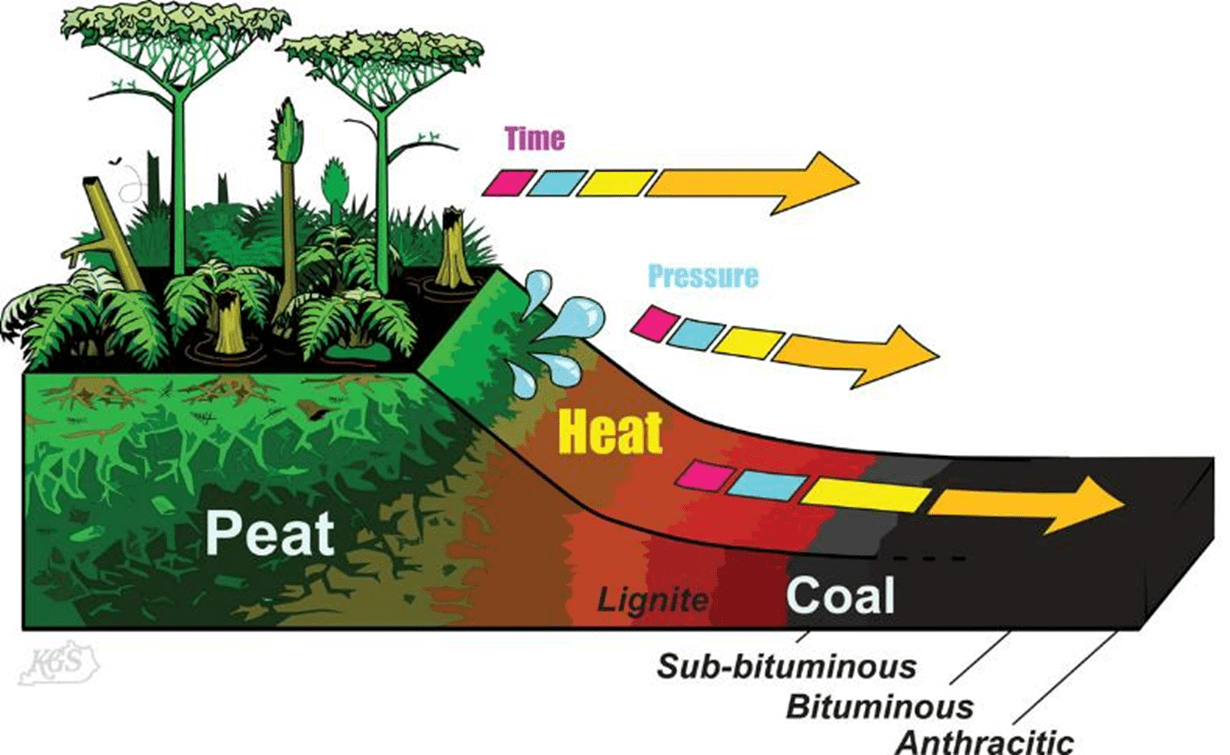
To prevent a peat drying out, the rate of peat accumulation must be matched by either a rising in the level of the water in the peat swamp or subsidence of the surface that the peat is growing on. Under optimal conditions peat grows at about 3 mm per annum. It takes about 20 metres thickness of peat to produce 1 metre of bituminous coal. A 6 metre thick bituminous coal seam will be formed from about 120 metres thickness of peat. This would take about 40,000 years of continuous peat accumulation to achieve this thickness of coal.
Based in this observation it is clear that the conditions for the formation of peat are:
-
- A very stable environment in terms of climate,
- Consistent subsidence (relative) of the land surface
- Long periods of time between flooding events.
The water table is required to be rising at about 3 mm/year for 40,000 years and there should be no rivers encroaching on the peat swamp.
Once the peat has formed, the peat needs to keep subsiding until it has reached up to 5 – 6 kilometres depth. During this process of increasing depth of burial, the weight of the sediments deposited on top of the peat initially squeezes the water out of the peat and the process that started with peatification (the formation of peat by bacterial and fungal action) is replaced by coalification which becomes dominated by thermal decomposition processes as the rock temperature increases from 10 – 15°C through to 160 – 180 °C. This burial process will be measured in million of years. During this process the coal seam will initially lose water (due to compression from the weight of the sediments above the seam) and then it will begin to lose carbon dioxide and methane along with the water as ground temperature increases. Once surrounding temperatures exceed 120 °C the coal will evolve methane and liquids (the source of oil and gas in many oil and gas fields). The eventual rank of the coal is a function of the final temperature that the coal is subjected to and the time the coal remains at that maximum temperature.
Where Does Coal Form
All organic substances oxidise or are consumed by bacteria and fungi if not preserved under anaerobic conditions. To preserve any peat that is formed requires that the region is undergoing steady subsidence. The most common tectonic setting that produces extensive peat swamps are associated with volcanic arcs in which a retro-arc basin forms on the continental side of the volcanic arc. The subduction of oceanic crust results in a tensional environment forming on the continental side of the volcanic arc. The tensional environment produces subsidence of the land surface which allows for the accumulation of peat Figure 1.
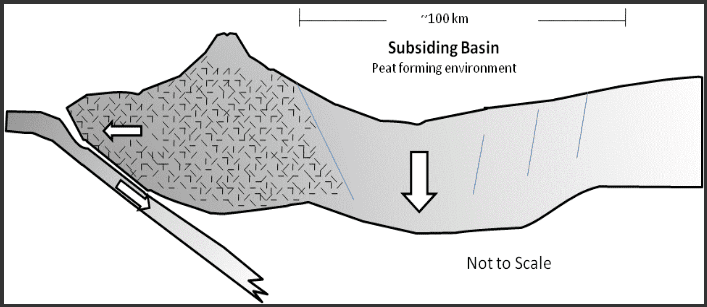
Figure 1: Back Arc Basin Coal Forming Environment
Subsidence in these environments is on-going for many millions of years
Coal Types
The various types of plant material that comprise the initial peat plus the post depositional actions upon the peat control the coal type. The post depositional actions can include air fall tuffs from volcanic activity, import of clastic sediments caused by flooding, drying out of the peat caused by climactic changes, fires within dried-out peat and submersion of the peat swamp. Permian coal seams from Australia, South Africa and India typically have bright coal at the base of the seam that grades into dull coal at the top of the seam. This is not a feature of tertiary age coal from Indonesia, New Zealand and Japan where coal seams are more uniformly bright caused by the action of anaerobic bacteria.
In macroscopic form the coal type is described simply as bright coal, banded coal and dull coal. Microscopic evaluation of these macroscopic forms shows they comprise three main organic types and one mineralogical type. The microscopic elements are known as macerals and are divided into 4 groups as shown below in Table 2.
Table 2: Coal Macerals
Maceral Group | Description | Additional Information |
Vitrinite | The vitreous part of coal that is normally represented as bright bands |
This maceral group has been formed from woody material. It has a low ash content and is responsible for the coking properties found in coals of coking coal rank. This maceral group is the dominant maceral group found in coals formed in the Tertiary and Cretaceous Periods. |
Liptinite | Comprises the remains of resin bodies, spores and leaf cuticles from plants. |
This group of macerals, is virtually devoid of ash and produces large quantities of volatile matter when heated. It can contribute to coking properties. |
Inertinite | Comprises part of plants that have been burnt, or oxidised by fungal attack |
This maceral group comprises a large part of Carboniferous and Permian coals. It is normally associated with higher ash content and when associated with a coking coal produces a stronger coke. |
Mineral Matter | Comprises primary non-organic minerals such as clays or quartz or secondary minerals such as calcite or pyrite |
This maceral group produces most of the ash found in a coal. Where the ash is not part of the plant material and has been either blown or washed in to the peat swamp it is mostly refractory and when it has been produced by interaction of carbonate and sulphate minerals it produces a coal ash that melts readily. |
Examples of the various maceral groups are shown in Figure 2 from an Australian Permian age coal from the Gunnedah region (Inland NSW). The picture shows the very bright inertinite at the top of the picture, the more variable (in composition) liptinite region in the middle of the picture and the clean grey vitrinite at the bottom of the picture.
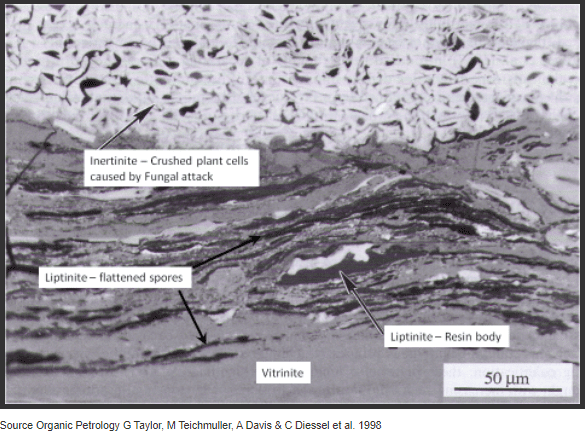
Figure 2: Coal Macerals from Permian Gunnedah Coal
Effect of Climate on Coal Type
Peat formation requires that the accumulation of plant material in the peat swamp must exceed the rate at which the actions of bacteria and fungi are consuming the plant material. Cellulose decomposing bacteria are most active at temperatures in the range of 35 – 40°C. Peat accumulated under elevated temperature conditions is normally degraded to a partially homogenised coal that is devoid of the characteristic banding.
Permian age coal seams from the former Gondwana Land (Australia, South Africa, Madagascar, India and Antarctic) were all formed under cool/cold conditions. The cold conditions slowed the rate of fungal and bacterial activity to the extent that in some conditions allowed for peat to accumulate at a faster rate than the subsiding land surfaces. Over time this faster accumulation of plant material would have caused the peat to be exposed to atmospheric conditions for longer periods of time allowing aerobic bacteria to degrade the plant material. A consequence of this exposure of the top of the seam to aerobic action is that the top of the coal seams comprise mostly inertinite macerals resulting from peat fires and aerobic bacterial and fungal action while the base of the seams are inertinite poor. Jurassic, Cretaceous and Tertiary age coals were formed under warmer conditions and fungal and bacterial activity was much greater causing the relative peat accumulation to match subsidence resulting in peat accumulating in wetter and more reducing conditions where anaerobic bacteria proliferated.
Superimposed on the climactic conditions influencing coal type has been the evolution of vegetation over time which has also had an impact on coal type. Coal has been formed in significant amounts over the last 360 million years and the major coal forming periods are shown below Table 3.
Table 3: Coal Basins Age & Characteristics
Age | Coal Basins/Countries | Climate/Dominant Plant Types |
Carboniferous (260 – 290 MY BP) | Kuzbass (Russia), Donets (Ukraine), Saar-Lorraine, UK/French coal fields,Polish and Czech coal fields Appalachain coal fields, Cape Bretton/Newfoundland coalfields, Mongolia, Korea | Warm moist climate, coal made from Lycopods (Lepidodendron and sigolaria). Gymnosperm (Cordaites) and Cycadophytes |
Permian (290-251 MY BP) | Australia, South Africa, India, Madagascar, Antarctica, Zimbabwe, China, Mongolia, Eastern USA, Canada | Climate considered to be cold with warm wet summers and freezing winters. Main plants Glossopteris and Gangangopteris |
Triassic (251 – 205 MY BP) | Australia, Vietnam, Mongolia, South America, China, western USA | Warm climate, low rainfall, similar plants to Permian |
Jurassic (205 – 141 My BP) | Australia, Russia, Mongolia, Scotland, Canada, China, South America | Appearance of flowering plants such as Angiosperms, gymnosperms and cycads still present |
Cretaceous (141 – 65 MY BP) | Canada, Western USA, Spitzbergen, South America, Mongolia, China, Indonesia, New Zealand | Cool to warm climate, angiosperms predominate |
Tertiary (65 – 1.78 MY BP) | Germany, USA, Japan, Australia, Indonesia, Thailand, Taiwan, China, New Zealand | Warm Climate angiosperms predominate |
Coal Rank
Coal rank is a measure of the progress of thermal maturation of organic matter deposited into a peat swamp along a path of increasing carbon content. The initial breakdown of the plant material by fungi and bacteria results in a lot of moisture being incorporated into the peat and consequently the energy content of the peat is lower than the plant components that initially comprised the peat. By the end of the peatification process, as the weight of sediments on top of the peat squeezes out water, the energy content of the peat increases. Beyond the peatification process the organic matter comprising the peat is subjected to increasing temperature as the coal is buried more deeply, the increasing temperatures cause condensation reactions to occur in the coal where carboxyl and hydroxyl components are expelled initially in the form of carbon dioxide and moisture and later in the form of methane and some liquids. This process continues through to the end of the bituminous rank. During this process, the carbon content of the coal increases as the oxygen content of the coal decreases. As the oxygen in the coal is removed the energy content of the remaining coal rises and coking properties are developed where the ash content of the coal is low enough. The development of coking properties occurs during the bituminous rank of the coal.
The progress of rank increase is shown in Figure 3 where the characteristics of each stage in the progression of rank from peat through to graphite is shown. In this figure the rarely found end members of the rank progression (meta-anthracite and graphite) are included.
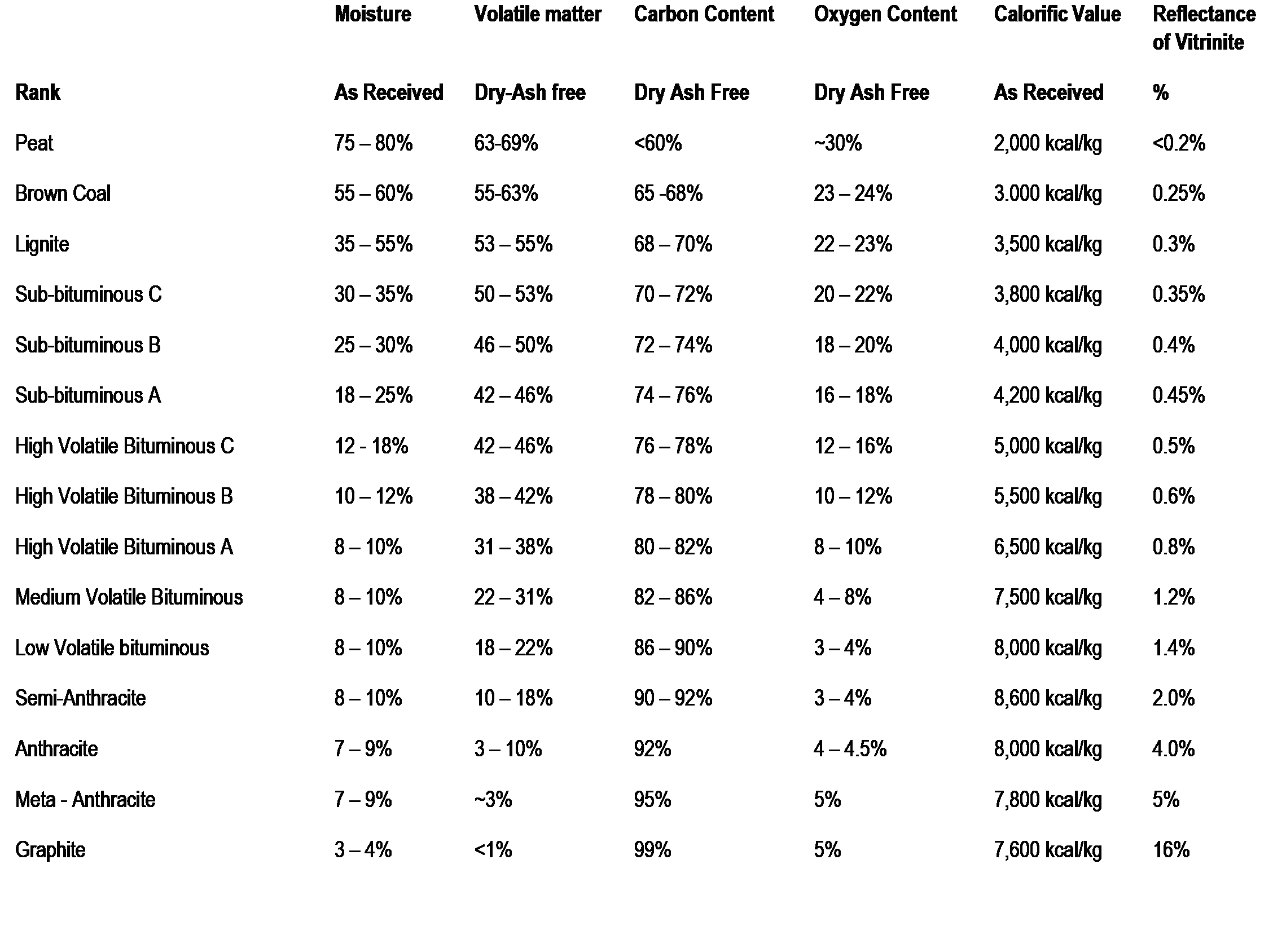
Figure 3: Coal Rank Progression
Usage of Coal
On the most basic level coal comprises moisture, volatile matter, fixed carbon, some sulphur and ash. Only the organic compounds in the coal contain energy and they are, the volatile matter, fixed carbon and the organic sulphur. The organic components of the coal comprise 5 elements, carbon, hydrogen, nitrogen, oxygen and sulphur. As the rank increases the carbon content of the organic matter increases and the oxygen content decreases. When oxygen content drops below about 12% coking properties can begin to develop. This development is promoted when oxygen cross linkages are broken and the breakdown of these cross linkages allows the remaining organic matter to respond to sudden heating by becoming plastic. The coking range finishes when the carbon content of the organic matter rises above 89 – 90% on a dry ash free basis. By this stage there is insufficient volatile matter left in the coal to develop the softening and swelling required for the formation of a well bonded and vesiculated coke.
Thermal Coals
All coals can be used for the thermal energy held in the hydrocarbons that comprise the organic portion of the coal. The energy is released when the organic matter in the coal is exposed to oxygen at high temperatures and is combusted in exothermic reactions. Where coals have a high moisture content, the moisture displaces the organic matter in the coal resulting in a lower energy content of the coal. Significant quantities of the contained energy are also used in evaporating the moisture. Where coals contain a high ash content the energy content is reduced in the same ratio as ash in the coal.
Since most thermal coals are used as a cheap source of energy, the inclusion of moisture and ash dilutes the energy content of the coal and consequently reduces the coal’s competitiveness in terms of $/GJ of energy. Where a customer is prepared to pay a higher price per GJ of energy, a coal producer can wash the coal to remove unwanted ash. The increased value of reduced diluting material is shown in Table 2.
Table 4: Reducing Contaminants and Increasing Energy Content and Value
Coal | Low Energy | Medium Energy | Higher Energy | High Energy |
Ash + Moisture content (ar) | 37% | 32% | 22% | 10% |
Calorific Value GJ/tonne | 15.91 | 17.60 | 20.94 | 24.72 |
Coal Value US$/tonne* | $78.05 | $94 | $148 | $200 |
$/GJ | $4.90 | $5.34 | $7.06 | $8.09 |
* Values from Platts ICT 20th April 2022
There is a cost to reducing the ash content of a coal through the washing process. The costs of washing the coal are incurred by all the coal washed but are borne only by the coal sold. To determine whether a coal is worth washing the increase in profitability of the coal mined is used to determine whether washing is worth the effort. In this exercise the total cost of each product obtained by washing the low energy coal is compared with the untreated low energy coal and ultimately the profit per tonne mined. As a lower ash coal is produced in each of the successive washing examples the cost of washing increases as more sophisticated means of reducing the ash are employed. Consequently, the cost of washing increases and the recovery decreases as higher energy products are produced (Table 5).
Table 5: Coal Washing Example
Low Energy | Medium Energy | Higher Energy | High Energy | |
Tonnes Mined | 100,000 | 100,000 | 100,000 | 100,000 |
Cost to CPP | $2,375,000 | $2,375,000 | $2,375,000 | $2,375,000 |
Washing Cost | $0.00 | $600,000 | $675,000 | $800,000 |
Total Cost | $2,375,000 | $2,975,000 | $3,050,000 | $3,275,000 |
Recovery | 100% | 85% | 70% | 58% |
Tonnes Sold | 100,000 | 85,000 | 70,000 | 58,000 |
Sale Price | $78.05 | $94.00 | $148 | $200 |
Income | $7,805,000 | $7,990,000 | $10,360,000 | $11,600,000 |
Profit | $5,430,000 | $5,015,000 | $7,310,000 | $8,425,000 |
Profit/tonne mined | $54.30 | $50.15 | $73.10 | $84.25 |
A prudent producer would wash the lower energy coal to the Higher Energy or High Energy products, but not the Medium Energy product.
All coals can be used for their energy content which can be captured and converted into electrical energy when used to generate electrical power. However much of the time the value of a coking coal far exceeds the value of a thermal coal and as a consequence thermal coals tend to be lower rank coals with little or no coking properties. Factors that impact the utility of coals for thermal coal uses are shown below:
- Free moisture in the coal, this affects handling of the coal (impedes its free running characteristics).
- Total moisture of the coal – this impacts the drying capacity of the grinding circuit.
- Hardness of the coal – this affects the mill power requirements to grind the coal to a size in which 70% is less than 75 microns.
- Ash fusion characteristics – normally the higher the temperature at which the ash fuses (melts) the lower the build-up of deposits on steam tubes that tend to reduce the efficiency of heat transfer into the water inside the steam tubes.
- Volatile matter – this is the most readily combustible part of coal and high volatile matter coals release large quantities of gases and tars leaving behind particles of char. The char takes longer to completely combust and the higher the rank of the coal the harder it is to combust the char completely. High rank (low volatile matter) coals require special combustion zone configurations to ensure complete burnout of the char particles.
-
Sulphur content – sulphur when burnt produces sulphur dioxide which can form acid rain. Sometimes sulphur is captured when carbonate minerals are in the coal ash capture sulphur oxide to form sulphate minerals such as gypsum. Some electrostatic precipitators (used to remove ash from the exhaust gases) work more efficiently when there is some sulphur dioxide in the exhaust gases.
- Ash content – should generally be lower than 30% as it is a diluent and increases weight for no benefit, it increases wear on grinding machinery and can cause inefficiencies in power station boilers. Coal ash often has pozzolanic properties (is cementitious) and can be added to cement to enhance workability of the cement, increase density of the concrete, reduce shrinkage, add strength as a result of the reduced shrinkage and reduce heat produced during setting.
- Specific Energy – the energy of coal is contained in the organic components of the coal including the volatile matter, fixed carbon and sulphur (organic and sulphide). Moisture and ash are ballast materials that increase the costs of transporting, drying and grinding of the coal. Specific energy of a coal can be described in four different ways:
-
- Dry basis (db) – the energy of the coal is determined after the coal has been dried
- Air Dried Basis (adb) – the energy of the coal after it has been dried under standard conditions of humidity and temperature
- As-Received Basis (arb) – the energy of the coal including all the moisture and ash it is delivered with
- Net As-Received (nar) – The energy available after the energy required to dry and coal and to drive off moisture produced from the combustion of hydrogen in the coal.
-
The conversion from gross as-received energy to net as-received energy requires the hydrogen content on an as-received basis and the moisture content of the coal (as-received) to be known. The conversion formulae varies depending on the method for expression of the energy content of the coal. Much international trade is conducted using kcal/kg as opposed to the metric system of energy description which is to use MJ/kg. The two short-cut methods to convert gar to nar are shown below:
Net as-received Energy Kcal/kg = Gross as-received Energy kcal/kg – 50.7 x Hydrogen (ar) – 5.83 x Moisture (ar). A coal with 6,322 kcal/kg gar, 3.8% hydrogen and 9% moisture the net calorific value can be calculated as Net Calorific Value = 6,322 kcal/kg – 50.7 x 3.8 – 5.83 x 9 = 6,076 kcal/kg nar.
Net as-received Energy MJ/kg = Gross as-received energy MJ/kg – 0.212 x H (ar) – 0.024 x Moisture (ar). The same coal with 26.47 MJ/kg, 3.8% hydrogen and 9% moisture the net energy content is as follows: Net Specific Energy MJ/kg = 26.47 – 0.212 x 3.8 – 9 x 0.024 = 25.45 MJ/kg
Metallurgical Coals
Metallurgical coals are used ultimately for their carbon content which is used to reduce a metal oxide to a metal
C + MO → M + CO2
Where C = Carbon, MO = Metal Oxide, M = Metal and CO2 = Carbon dioxide gas
There are two types of metallurgical coals, coking coals and non-coking coals. Coking coals are those coals with a low ash content that upon rapid heating in the range 350 – 550°C soften and swell then on further heating above 500°C give off tars and gases leaving behind a solid, hard, porous, carbon residue known as coke. The coals used to make the coke typically have a low ash content (8 – 10.5%), a moderate vitrinite content ~50% and a low sulphur content (<0.6%). The suitability of a coal for the coking process is determined by the reflectance of the vitrinite component of the coal. For the coke making industry the reflectance for coking coals ranges from 0.6% to 1.8% reflectance. Coals with the lowest reflectance have the lowest rank and highest volatile matter and coals with the highest reflectance have the highest rank and lowest volatile matter. Reflectance measures the amount of graphitic crystallite within the coal. The graphitic structures comprise aromatic ring structures that in low rank coals are separated by aliphatic molecules and are generally randomly orientated. In higher rank coals the aliphatic ring structures are much fewer in number and generally shorter chained and the aromatic ring structures are more aligned. The light bounces off the ring structures and is absorbed by the aliphatic components of the coal (Figure 4).
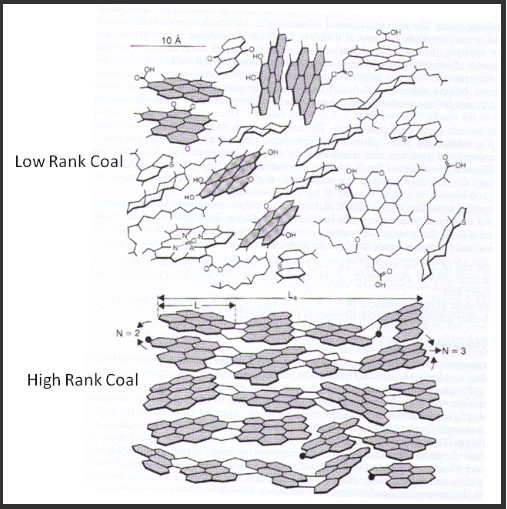
Figure 4: Carbon Structures Influencing Reflectance
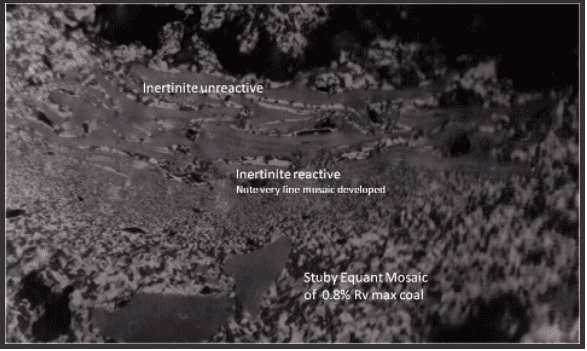
Figure 5: Stubby Equant Mosiac from a 0.8% Reflectance Coal
The graphitic crystallites are the precursors of mosaics (carbon structures) observed in cokes. In lower rank coking coals the mosaics formed are fine grained and disordered, while in high ranking coking coals the mosaics formed are large and show some similarities to graphite. Where ash chemistry is the same the reactivity of the coke to CO2 is related to the size of the mosaic formed on coking. Three examples from the Northern Bowen Basin are shown below where coke mosaics from coals with 0.8% reflectance, 1.0% reflectance and 1.3% reflectance are shown. The first photomicrograph shows a fine stubby “equant” mosaic at the bottom of the picture grading up through fine to very fine mosaic as a maceral of inertinite is approached. The coal would be classed as a semi-soft coking coal.
A coke from a 1% reflectance coal is shown in Figure 6 where flame mosaic is produced. The coal would have been classed as a soft coking coal.
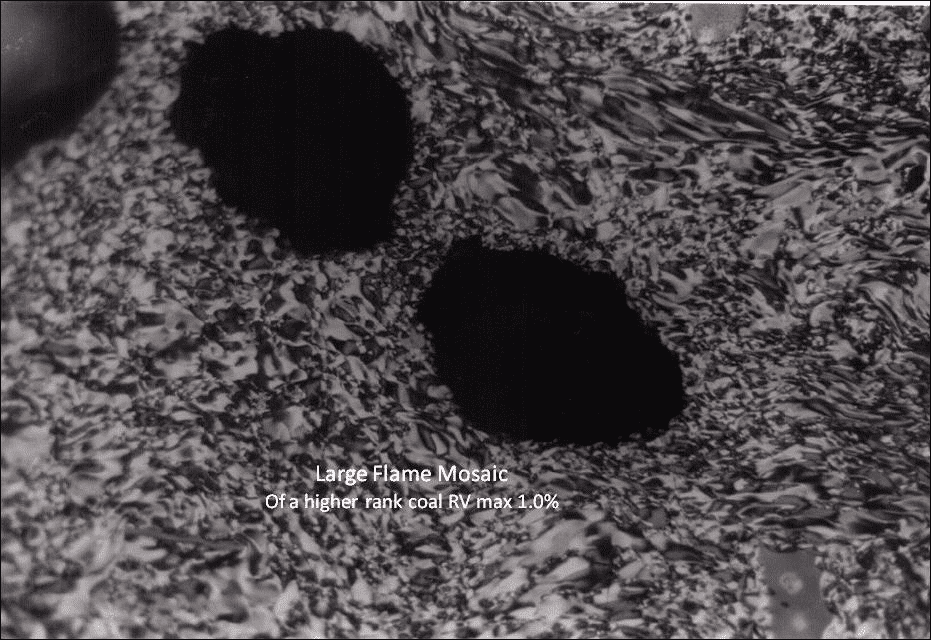
Figure 6: Flame Mosaic from 1.0% Reflectance Coal
As the rank of the parent coal increases the size of the mosaic increases and this is shown in Figure 7 where large ropey mosaic can be seen with much smaller vesicles that result from a much lower volatile matter coking coal. This coal would be classed as a hard coking coal.
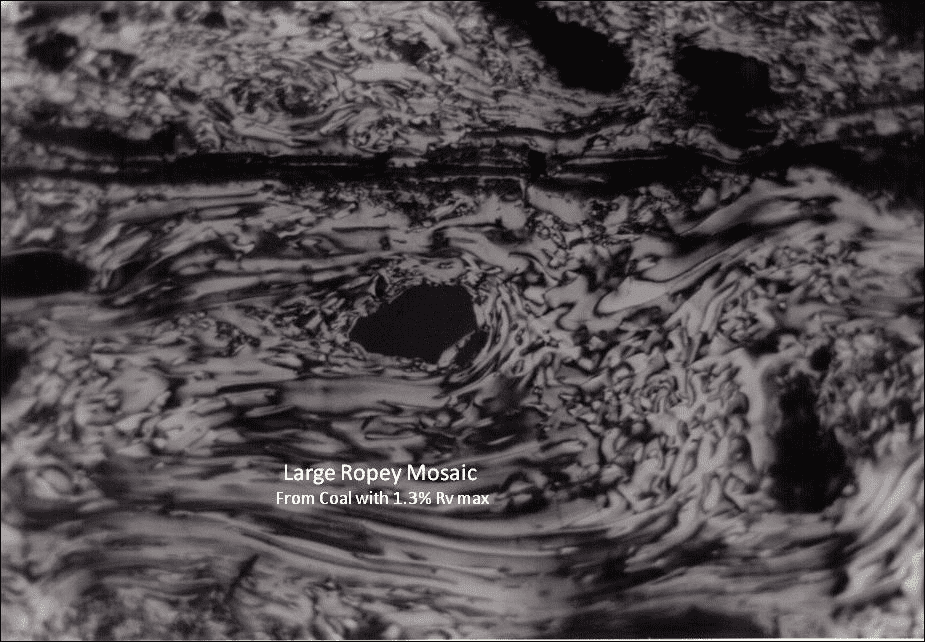
Figure 7: Ropey Mosaic from a coal with 1.3% Reflectance
The size and type of mosaic produced in the coke mostly determines the reactivity of the coke to CO2. This feature is important in modern large blast furnaces used for the reduction of iron ore to molten iron. The coke is used for three major purposes:
-
- Carbon for reducing the iron oxide to iron
- As an open supporting structure to provide a pathway for post reaction gases to exit the blast furnace
- Energy for driving the endothermic reduction reactions in the blast furnace through the combustion of the coke at the bottom of the blast furnace.
The reduction reactions follow a 2 step process whereby carbon from the coke reacts with CO2 released from the combustion of the coke at the bottom of the blast furnace. The coke reacting with the CO2 produces carbon monoxide which is a potent reducing gas able to penetrate the iron oxide lumps and react with the oxygen in the iron oxide to produce iron plus CO2 gas. The coke should be sufficiently unreactive so as not to react with CO2 at temperatures below 1,100° C. Where the coke reacts with CO2 at temperatures below 1,100°C the carbon monoxide produced will be wasted as the blast furnace temperatures will be too low to allow the carbon monoxide to reduce the iron ore. This reaction is called the carbon solution reaction and is shown below:
Carbon (from Coke) + CO2 → 2CO
Two factors influence the speed of the carbon solution reaction:
-
- The type of mosaic – long ropey mosaic has few edge groups for the carbon solution reaction to act on, finer, more equant mosaics have many edge groups that can partake in the carbon solution reaction.
- The chemistry of the ash – it has been found that cokes with alkaline ash chemistry catalyse the carbon solution reaction and cokes with ash dominated by the elements silica and alumina remain unreactive until high temperatures are reached.
Coke makers and blast furnace manufactures measure the ability of a coke to resist the carbon solution reaction and report the performance as the Coke Strength after Reactivity (CSR). Cokes with a CSR above 66 are considered to be the best for use in Jumbo Blast furnaces while cokes with CSR of 60 are considered to be good and suitable for normal large blast furnaces while cokes with CSR of ~50 are suitable for small blast furnaces.
PCI
Modern blast furnaces use high quality coke in combination with pulverised coal injection (PCI) injected at the bottom of the blast furnace. The coke is primarily used to provide the permeability within the blast furnace while using PCI for the heat and as a source of CO2 at the bottom of the blast furnace. Currently low volatile matter PCI coal is selling for US$460/tonne FOB and coke currently has a value of ~US$700/tonne for high CSR coke making PCI replacement of coke a worthwhile exercise. The PCI has about the same calorific value as a metallurgical coke and the ash content at about 8.5% is lower than a metallurgical coke with ~11% ash. The added benefits are extended to a reduction in the amount of slag that is required to be melted and disposed of by the blast furnace operators
Coke Manufacture
The best coking coals produce a coke with CSR of 74, but are restricted to just a few mines around the world. Most of these mines are in Queensland Australia. Manufacturers of coke use many methods to reduce the cost of the coke while at the same time producing a coke with the required cold coke strength and required CSR. Use of coals with a lower CSR such as semi-soft coking coals, semi-hard coking coals can be offset through the use of various techniques used by coke manufacturers to enhance coke strength and to reduce the carbon solution reaction without having to resort to the use of high CSR coking coals. Use of higher volatile matter coals (generally found in semi-hard and semi-soft coking coals) causes increased shrinkage of the coke and this can lead to increased fissuring of the coke mass resulting in small coke lump size which results in greater coke reactivity and lower permeability of the blast furnace. To overcome these issues coking coal blends are stamp charged where the bulk density of the coking coal blend is increased through stamping to remove any gaps between coking coal particles, finely ground coke breeze (fines) are added to the blend (to reduce volatile matter and reduce shrinkage) and finally the coke oven blend is dried to allow for a much faster heating rate that promotes more plasticity and better bonding within the coke oven blend.
Where coke manufacturing is included as part of an integrated steel mill, the coke oven gas can be used for process heat and for generating electricity for use in the plant or sale to the national grid. The blast furnace is a source of carbon monoxide, some hydrogen and methane and this gas – called blast furnace gas has low calorific value but can be used with coke oven gas to power electricity production or provide heating in the rolling mills.
Non Blast Furnace Route for Metals Production
In India, South Africa, Korea, China and New Zealand, iron is produced from routes other than the blast furnace by use of direct reduction kilns. These kilns do not have a large weight of iron ore bearing down on the reductant and as a consequence non-coking coals can be used. Low ash coal that is reactive is charred in a multi-hearth furnace to remove volatile matter and produce a carbon rich char that is mixed with the iron ore which may be a briquette or a fine magnetite sand. The carbon acts like the carbon in a blast furnace where it reacts with CO2 to produce CO gas. The CO reduces the iron oxides to an iron sponge. The low rank coals used in direct reduction applications do not have the energy content to allow combustion temperatures in the rotary kilns to achieve the temperatures required to melt the reduced iron, these lower temperatures result in the production of a fused sponge iron. The sponge iron is melted in an electric arc furnace and converted into steel.
Copper and lead ores are primarily sourced as sulphide ores and the traditional process is to oxidise these ores by burning off the sulphur and making a metal oxide which is then reduced using coke in a furnace.
Production of silicon metal follows the process of using reactive forms of hard carbon to reduce quartz (silicon dioxide) to silicon metal. The production of aluminium follows a similar process where carbon anodes made from petroleum coke or meta anthracite are inserted into a pot filled with alumina and a high amperage current is applied which provides heat and forces the oxygen from the alumina to combine with the carbon from the anode to produce CO2 and molten aluminium.
SiO2 (quartz) + C (from sized coal) → Si Metal + CO2
Al2O3 (alumina) + C (from a baked anode) → Al (metal) + CO2
The chemical requirements for the coal when used to make silicon are very strict as the coal ash becomes incorporated into the liquid and can contaminate the silicon metal. Consequently, the coal ash must have low levels of iron, calcium and aluminium. The requisite levels of these contaminants are normally achieved through the use of very low ash coals (~1%) for the process. The maintenance of high reactivity of the coal is achieved through the use of low rank, and high volatile matter coals. Coals of this rank are normally harder and are more easily sized.
Other Uses of Coal
Activated carbon can be made from low ash bituminous coals and chars. The carbonaceous char/coke is activated by use of steam that generates active sites that have surface charges that attract molecules of chlorine, organic compounds and other pollutants that are required to be removed from fluid streams. Activated carbons can be used to remove molasses from sugar syrup to make white sugar.
Anthracites are coals that have been metamorphosed through exposure to elevated temperatures and pressures for a long period of time. Where the anthracites have a low ash content, the carbon content of the coal resembles that of a coke as does the volatile matter content. Anthracites do not have the physical strengths of a coke and so can be used in applications for which strength is not a requirement and where the anthracites are hard enough to be sized they can be used in water filtration to scavenge clays from the water as well as chemical species. Meta-anthracites – those anthracites with <3% volatile matter can be used for the manufacture of carbon anodes and cathodes as they have good conductivity and good abrasion resistance. Anthracites have been used extensively as smokeless fuels as they have very little volatile matter (which is the source of most black smokes). The very low volatile matter generally renders anthracites difficult to ignite.